Introduction
The escalating global demand for food, compounded by a burgeoning human population, underscores the pressing need for sustainable solutions. Aquaculture emerges as a key player in addressing this challenge, offering a promising avenue to enhance food security while minimizing environmental impact. This article explores how aquaculture, with its resource efficiency and alignment with the Sustainable Development Goals, stands as a beacon for securing global food supplies.
Biotoxins in aquaculture
Nevertheless, the aqua industry encounters a formidable challenge in the form of biotoxins, which pose inherent threats to fish, shrimp, and consumer health. Biotoxins are substances characterized by their toxic nature and biological origin. They manifest in various forms and can be produced by a wide array of living organisms (fungal biotoxins or mycotoxins, algal and bacterial toxins), plant toxins, or phytotoxins, and animal toxins (Picardo et al., 2019).
Mycotoxins
Mycotoxins are toxic substances produced by certain molds (fungi) that can contaminate food and feed, including those used in fish and shrimp aquaculture. The presence of mycotoxins in aquatic species can have detrimental effects on both the health of the shrimp and the consumers, who ultimately consume the contaminated products.
The potential sources of mycotoxins contamination in aquaculture include the feed provided to them, as well as the water and environment in which they are raised. Mold growth on feed ingredients, especially in humid or poorly stored conditions, can lead to mycotoxins production. Additionally, mycotoxins can be present in water and sediments, posing a risk to aquatic organisms.
Other biotoxins
Although major attention has been given to mycotoxins, other biotoxins, such as marine toxins, also require thorough investigation. Marine toxins are classified as natural toxins produced by certain marine organisms, including algae, bacteria, and invertebrates. The classification of marine toxins encompasses various types, each originating from different marine sources:
- Phycotoxins: Produced by harmful algae (phytoplankton), phycotoxins include toxins such as okadaic acid, saxitoxins, brevetoxins, and domoic acid. They are associated with harmful algal blooms (HABs) and can lead to shellfish poisoning when contaminated seafood is consumed.
- Ciguatoxins: Originating from certain species of dinoflagellates, ciguatoxins accumulate in larger predatory reef fish. When humans consume fish containing ciguatoxins, it can result in ciguatera fish poisoning, characterized by neurological symptoms.
- Tetrodotoxin: Produced by bacteria and found in pufferfish and other marine species, tetrodotoxin is a potent neurotoxin. Consumption of fish containing tetrodotoxin can lead to tetrodotoxin poisoning, affecting the nervous system.
- Dinoflagellate Toxins: Dinoflagellates are responsible for producing toxins such as brevetoxins, which can lead to neurotoxic shellfish poisoning (NSP) when contaminated shellfish are consumed.
Among the approximately 5000 known species of phytoplankton, around 2% are capable of producing toxins that exert a significant impact on marine communities, as highlighted by Hallegraeff (2014). Predominantly associated with the dinoflagellate taxa, these organisms can instigate toxic events even at relatively low concentrations, posing a considerable threat to both human health and the marine ecosystem.
Biochemically, these biotoxins represent secondary metabolites with a diverse array of effects, as outlined in Table 1. They can influence the nervous system, leading to enduring short-term memory loss in the case of domoic acid or causing sensorimotor impairment that may culminate in fatality due to paralytic shellfish toxins. Additionally, these toxins can impact the digestive tract, eliciting symptoms of gastrointestinal distress. The pervasive nature of these biotoxins underscores their potential for far-reaching consequences in marine environments and human well-being.
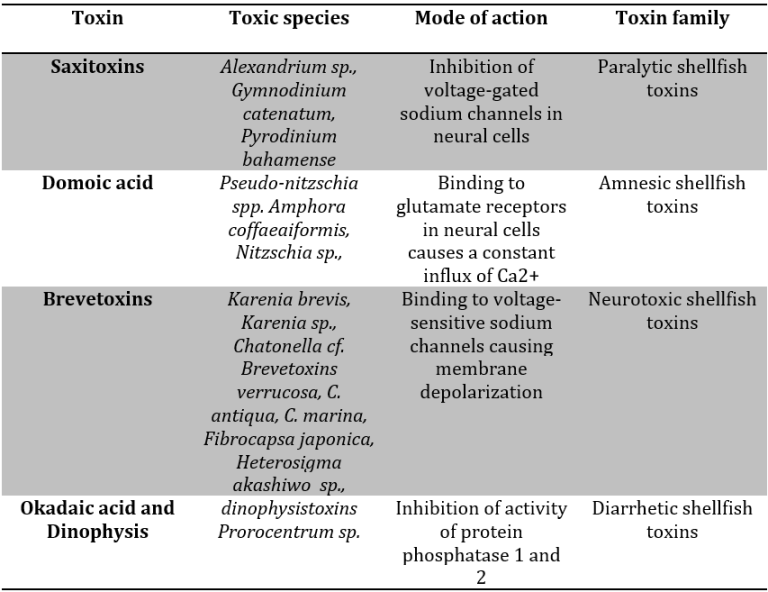
Table 1: The most common toxins produced by marine phytoplankton.
Harmful Algal Blooms (HABs) not only impact marine organisms but also pose significant risks to human health, as highlighted by Costa et al. (2017), and entail socio-economic consequences and costs, as discussed by Visciano et al. (2016). The direct effects on human health can manifest through consuming contaminated seafood, skin exposure to contaminated water, and/or inhaling aerosolized biotoxins (Visciano et al., 2016). These biotoxins can be categorized into water- and fat-soluble molecules, each causing distinct symptoms. Water-soluble toxins may lead to Paralytic Shellfish Poisoning (PSP) and Amnesic Shellfish Poisoning (ASP), while fat-soluble toxins can result in Diarrhetic Shellfish Poisoning (DSP) and Neurotoxic Shellfish Poisoning (NSP) (FAO/IOC/WHO, 2004; Visciano et al., 2016).
Consequently, the contamination by marine biotoxins has emerged as a significant global concern for public health authorities and the aquaculture industry, underscoring the need for comprehensive monitoring and management strategies (Liu et al., 2019). Contemporary evidence suggests that the combined impacts of eutrophication and climate change may elevate the likelihood and severity of HABs (Pavagadhi et al., 2013).
Planktivorous filter-feeders among small marine pelagic fish, such as sardines and anchovies, have been identified as potential vectors of marine biotoxins, specifically domoic acid, within the trophic chain during toxic algae blooms (Lefebvre et al., 2013; Costa et al., 2004). Instances of poisoning outbreaks resulting in substantial mortalities among marine top predators, including marine mammals and seabirds, have been globally reported after the consumption of small pelagic fish containing elevated levels of marine biotoxins, such as saxitoxin and its derivatives (Costa et al., 2016).
In addition to planktivorous filter-feeders, fish may encounter marine toxins through extensive feeding on bivalves that harbour significant amounts of HAB toxins. Notably, Okadaic acid (OA) and related toxins constitute frequently occurring groups of marine toxins in southern Europe, Asia, and South America (EFSA, 2008). These toxins tend to accumulate prominently in bivalve molluscs, particularly in species like mussels, where they can persist in tissues at elevated concentrations for extended periods (Vale, et al., 20089; Rossignoli et al., 2011).
Routes of toxin exposure for aquatic animals
Routes of toxin exposure are shaped by three pivotal factors: the ecology of the toxin producer (e.g., pelagic or epibenthic), the environmental conditions and the likelihood of organisms encountering the toxin. Toxin transfer can transpire through either the foodborne or waterborne pathway, with toxicity manifesting along the food web or from toxins dissolved in the water after cell release or excretion.
Ingestion of toxic phytoplankton cells by filter-feeding organisms, including bivalve mollusks, zooplankton, and planktivorous fish, results in the accumulation of toxins inside the cells in the predator’s viscera. This initiates a cascade of vectors throughout the food web, potentially causing adverse effects in marine communities and reaching apex predators. Filter-feeding organisms, like molluscs, are frequently identified as the primary vectors for human bio-intoxication. The persistence of toxins in the water is influenced by the physical and chemical properties of seawater and the volume of water where the toxin has been released. Larger water volumes tend to dilute the toxin, mitigating the risk of contamination.
The effects of marine toxins on aquatic species
The effects of biotoxins on aquatic species’ early development can vary depending on the stage of reproduction, including exposure during sexual maturation, as eggs, and as larvae (Vasconcelos et al., 2010). The impact of a single toxin differs across these phases due to variations in exposure levels and the pathways through which the toxin reaches its target. Contact with toxins can occur directly, through feed ingestion, or during the collapse of phytoplankton blooms when toxins dissolve in the environment.
Fish were found to remain contaminated for short periods, particularly during the mid-bloom period, accumulating 56.2 µg kg-1 of its free form (Alves and Mafra, 2018). Another study reported the presence of okadaic acid (OA) at low levels (44 ng g-1) in a pool of liver and digestive tract tissues from the filter-feeder fish Cetengraulis edentulus in Paranaguá Bay (Mafra et al., 2014).
Investigations into the effects of biotoxins in fish under controlled conditions have revealed significant impacts (Table 2). Ajuzie (2008) demonstrated that OA exposure to sea bass juveniles could affect their survival, potentially altering fish behavior and impacting vital organs. Histological examinations revealed no alterations in the kidney, stomach, and intestinal tissues of fish directly or indirectly exposed to P. lima. However, gill and liver tissues were highly impacted. Fish in contact with the cell-free medium of P. lima exhibited abnormal behavior, repeatedly jumping out of the medium due to inadequate oxygen passing over their gills caused by the high viscosity of the medium. Gill swellings and separation of lamellar epithelia from gill vessels affected gaseous exchange, leading to hypoxia and a cascade of events disrupting fish metabolic systems and causing death. P. lima was also found to induce fatal degeneration in liver architecture, including hydropic degeneration, hepatocyte hypertrophy, and dissociation, resulting in the loss of tissue integrity and functions.
Le Du et al. (2017) explored the impact of dissolved DSP toxin in Seriola rivoliana, revealing strong toxicity to embryos with mortality ranging from 60% to 100% at different toxin concentrations. In medaka fish embryos exposed to OA, retardation of embryo development was observed, leading to reduced survival rates, up to 100%, depending on the dose (Escoffier et al., 2007). Furthermore, Souid et al. (2018) demonstrated oxidative damage relevance for OA toxicity in Sparus aurata, a valuable fish species in the Mediterranean coast food web. They observed increased levels of oxidative damage markers, such as malondialdehyde (MDA), and pronounced histopathological changes in the liver and gills after OA exposure.
Additionally, Fladmark (1998) found that salmon hepatocytes exhibited higher sensitivity to algal toxins, particularly OA and DTX-1, which were ten times more potent as apoptosis inducers and aggregation inhibitors for salmon. The exposed salmon hepatocytes showed altered surface properties, indicating a lack of special intracellular resistance to common marine toxins at cytotoxic levels.
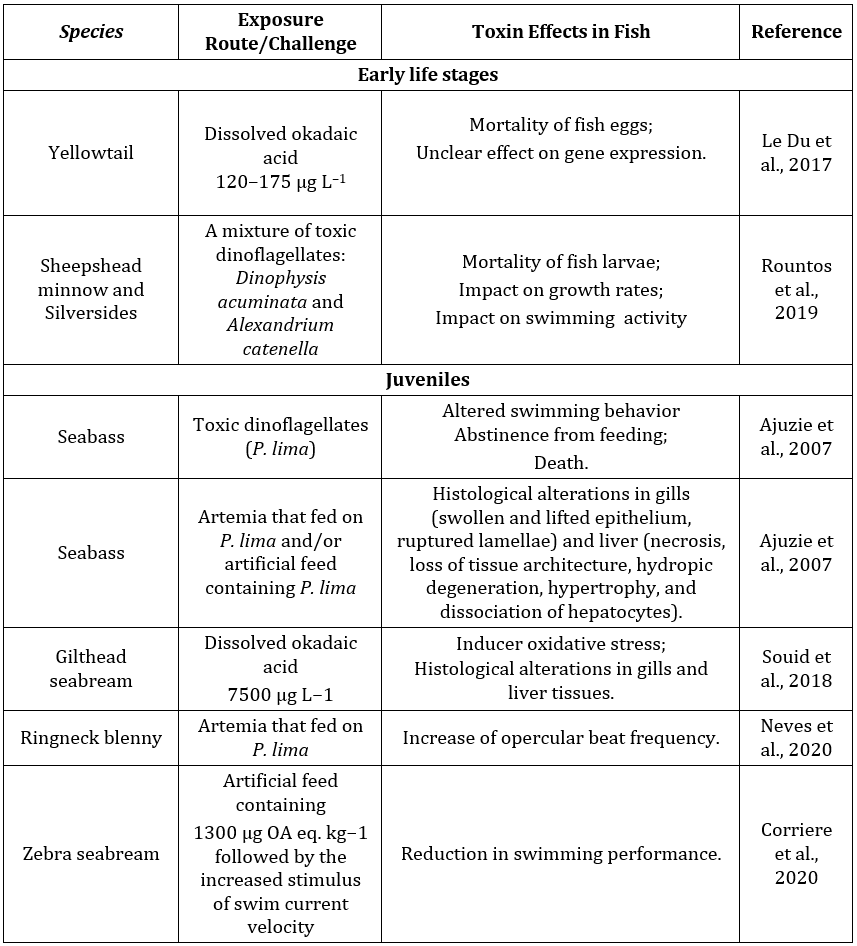
Table 2. Effects of some biotoxins in marine/estuarine fish
Biotoxin detection methods
Biotoxin detection methods have traditionally employed bioassay techniques, despite their drawbacks such as insensitivity, lack of specificity, and ethical concerns related to the use of animals, particularly mice. To address these limitations, alternative analytical methods for biotoxin detection, such as biosensors and chromatographic techniques, have gained prominence.
Biosensors represent advanced detectors extensively utilized with biological samples due to their cost-effectiveness, compact size, and portability. These devices operate based on the interaction between the target cell and a bio-receptor (e.g., DNA, enzymes, or cells) (Farag and Tanios et al., 2021). The resulting product undergoes a specific signal transformation that can be detected using transducers, including optical, electrochemical, or mass-based methods. Biosensors offer the advantage of easy modification depending on the target activity and serve as a preliminary screening tool for the presence of biotoxins before employing more sophisticated techniques (Neethirajan et al., 2018).
In the case of OA, an enzyme-based biosensor is employed to measure its inhibition activity, given that OA inhibits the phosphatase enzyme. Biosensors have proven valuable in detecting OA activity (Hamada-Sato et al., 2004), and their development for detecting biotoxins in crustaceans and other marine organisms is essential for assessing health risks.
For Paralytic Shellfish Poisoning (PSP) biotoxins, high-performance liquid chromatography (HPLC) coupled with highly sensitive detection units, such as fluorescence detectors or mass spectrometers, is commonly employed. The coupling of hydrophilic interaction liquid chromatography (HILIC) with electrospray ionization tandem mass spectrometry (MS/MS) has shown improved results in detecting PSP toxins. This approach not only enhances detection sensitivity but also provides structural elucidation capabilities to confirm toxin chemical structures (Galeano Garcia et al., 2019).
In a study along the Portuguese coast, HPLC-UV was used to determine domoic acid accumulation in swimming crabs in 2003. The study revealed a high rate of DA accumulation within the crab visceral tissues, exceeding the regulatory limit and highlighting the effectiveness of HPLC in detecting toxin levels(Costa et al., 2003). This underscores the importance of employing advanced analytical techniques for accurate and sensitive biotoxin detection in marine organisms.
Conclusion
As conclusion, the aquaculture industry endeavours to meet global food demands sustainably, the establishment of effective monitoring and management strategies for biotoxins becomes imperative. The intertwined challenges of climate change accentuate the urgency of ongoing research and adaptive measures to mitigate the adverse impact of biotoxins on aquatic species.