Introduction
This article describes the toxicokinetics of the most important mycotoxins within the pathology of production animals. The information presented provides an overview of the chemical properties of mycotoxins and their respective processes of absorption, distribution, metabolism and excretion in production animals and delves into the differences found between species.
Aflatoxins
Chemical properties
Aflatoxins have a common structure of four rings, a property which gives them a rigid and flat geometrical structure. The most important aflatoxins are B, G and M, which is a metabolite of the B. The difference between B and G lies in the six-lactone ring. Besides, the differentiation between classification 1 and 2 lies in the oxidation of the double bond. Regarding the other aflatoxins metabolites, aflatoxicol (AFL) is obtained by the oxidation of the ketone group, AFP1 is created when the ether group is converted to an alcohol, and in AFQ1 has an alcohol group in the cyclopentanone group.
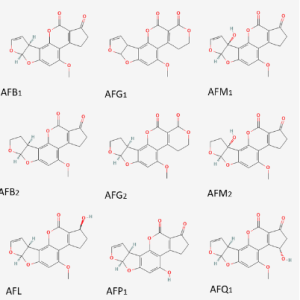
Figure 1. Chemical structure of the main aflatoxins and their metabolites.
Absorption
The main absorption of aflatoxin B1 occurs in the small intestine and largely depends on its molecular weight and lipophilic characteristics (Schrenk et.al, 2020).
Distribution
According to distribution, metabolism and excretion, the organ that is mainly affected by the effect of aflatoxins is the liver. Furthermore, it can also accumulate in the muscle (Popescu et.al, 2022).
Metabolism
Aflatoxin B1 is metabolized during its passage through the gastrointestinal tract, but the main site where it gives rise to its extensive metabolism has not yet been elucidated. Hepatic exposure to aflatoxin is closely related to the concentration of AFB1 and the absorption rate in the intestine, in addition to hepatic portal flow (Schrenk et.al, 2020).
In the liver, aflatoxins are substrates of CYP monooxygenases, including CYP3A4, 3A5 and 1A2. An important step in the activation of AFB1 is the formation of AFB1-exo-8,9-epoxide, which is its reactive form. Only AFB1, AFG1 and AFM1 can be activated by CYPs in such a reactive form. AFB1 has many other metabolites that are obtained by different reactions. For example, Aflatoxicol is a molecule that is obtained from the reduction of AFB1 through a NADPH-dependent reductase in the liver. Other important metabolites are: AFM1 and AFQ1 (obtained by hydroxylation), AFP1 (formed by the demethylation process) and the oxidation products (AFQ1 and AFM1). One of the important enzymatic processes that the body has to reduce the reactive form of AFB1 to a less reactive metabolite such as AFB1-dialcohol is carried out by the family of NADPH-dependent aldo-keto-reductases. Of the mentioned metabolites: AFM1, AFP1, AFQ1 and aflatoxin-dialcohol can be conjugated with glucuronic acid and excreted in feces or urine. Unlike AFB1, information on the metabolism of the other types of aflatoxins is limited, with only limited literature identified on the metabolism of AFB2 (Schrenk et.al, 2020).
Excretion
The excretion of AFB1 and its metabolites is mainly generated through the biliary route, followed by the urinary route. Lactating animals may excrete a fraction of the ingested AFB1 in the form of AFM1 and other metabolites through milk (Eaton & Groopman, 1994).
Deoxynivalenol
Chemical properties
Deoxynivalenol (DON) is a mycotoxin that belongs to the trichothecene family. Trichothecenes are characterized by having a common structure of three rings and an epoxide at carbon 12. Within this group of compounds there are four types: A, B, C and D. The most common types in nature are A and B. DON is a type B trichothecene which is characterized by having a ketone group at the 8-carbon position.
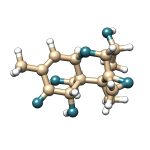
Figure 2. Chemical structure of deoxynivalenol (DON).
Absorption
Intestinal absorption and metabolism of DON varies widely among different domestic animals and depends greatly on regional pH, intestinal segments and bacterial activity. It is the microbial localization, which affects the bioavailability of DON and its metabolites when ingested (Knutsen, 2017). Moreover, DON absorption changes according to the animal species, age and even sex. In addition, the appearance of DON in the blood after oral ingestion is rapid for most mammalian species (Payros et.al, 2016).
Distribution
The distribution of this toxin occurs in multiple organs, without its concentration being uniform. After oral administration, DON has high values in plasma, liver and kidneys after 30 minutes and subsequently, its concentration becomes higher in the intestinal tract after 1 hour. This can be explained in the sense that DON is rapidly absorbed in the digestive tract and briefly circulates in the plasma, moving to multiple organs. By liver enzymes, DON is efficiently metabolized and excreted through urine, reducing its concentration in plasma, liver and kidneys. With this, unabsorbed DON remains in the digestive tract and since intestinal movement is much slower than plasma circulation, it accumulates. To end the distribution issue, DON has also been detected at low levels in the brain, suggesting the possibility of movement of this toxin across the blood-brain barrier, causing brain inflammation (Sun et.al, 2022).
Metabolism
The main metabolism of deoxynivalenol focuses on two main pathways: phase II metabolism and intestinal biotransformation. Phase II metabolism involves the formation of glucoside, glucuronic acid, and sulfate conjugates. On the other hand, biotransformation is related to the transition from DON to DOM-1. The use of both metabolic processes varies according to the species. For example, in pigs and humans, between 70 to 94% of DON is glucuronidated, while a small portion is detoxified by intestinal microbiota (Sun et.al, 2022).
Phase II metabolism is quite versatile in different animals. Birds usually manifest sulfate conjugation, while other animals use the glucuronidation pathway. A relevant fact is that pigs and humans share the metabolite DON-12GlcA (obtained by phase II metabolism), which is why this animal is used as a model for studying toxicokinetics and human metabolism (Sun et.al, 2022).
Intestinal transformation by bacteria from DON to DOM-1 is another important route for detoxification. In chickens, it is suggested that this metabolic pathway is one of the main reasons for their resistance to DON and although extensive evidence is not available, studies have confirmed that the toxin level decreases as it progresses through the intestinal tract of birds. However, DOM-1 is not the only metabolite product of biotransformation, but the transformation of DON into DON-3S has been detected in broiler chickens (Sun et.al, 2022).
Excretion
The excretion of DON and its metabolites in pigs, mice and humans occurs exclusively in the urinary tract; unlike chickens and rats, where the proportion of DON in feces can be higher than that found in urine (Sun et.al, 2022).
Zearalenone
Chemical properties
Zearalenone is a mycotoxin with a resorcyclic acid lactone structure. The main metabolites of zearalenone are α and β-zearalenol, which are generated by oxidation of the ketone group to an alcohol. α and β-zearalenol are isomers that differ in the chirality of the carbon to which the hydroxyl group is bonded.
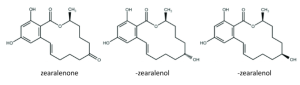
Figure 3. Chemical structure of zearalenone and its metabolites.
Absorption
Zearalenone is a mycotoxin with rapid and extensive absorption after oral administration in rats, rabbits, pigs and humans (EFSA, 2011). One of the particularities of zearalenone absorption is that after being absorbed by the intestine, part of ZEA returns through bile excretion for a second absorption (Han et.al, 2022).
Distribution
Zearalenone and its metabolites can be detected in both animal tissues and their products. The detection of ZEA is quite limited in the liver, kidney, muscle and plasma. It is its metabolites α and β-zearalenol that have a better distribution. It is worth mentioning that these metabolites have an enormous affinity for albumins, which is why they remain widely in the plasma (Liu & Applegate, 2020). Other organs involved are the reproductive organs (uterus, testicles and ovaries) and adipose tissue (Han et.al, 2022).
Metabolism
The metabolism of zearalenone has been investigated in vivo in both animals and humans. In consequence, three important biotransformation routes were reported in animals (EFSA, 2011).
First, there is the enzymatic reduction of zearalenone catalyzed by 3α and 3 β-hydroxysteroid dehydrogenase (HSDs), producing α and β-zearalenol and a small group of zearalanols. The primary reduced forms of the toxin have different estrogenic activities, and the toxic reactivity of α-zearalenol is greater than zearalenone and β-zearalenol. In mammalian species, hepatic transformation of zearalenone is completely different regarding the reduced metabolites and glucuronides. For example, pigs transform zearalenone mostly to α-zearalenol, while ruminants transform it into β-zearalenol, which explains a greater sensitivity on the part of swine (EFSA, 2011).
The second metabolic process is monohydroxylation. Reported in humans through cytochrome P450 (CYPs) and liver microsomes. Catechols are generated by aromatic hydroxylation and these metabolites are subsequently oxidized into quinones that develop a redox cycle and covalently modify biological macromolecules. The estrogenic properties of these catechols remain unknown (EFSA, 2011).
As the last metabolic process, the phase II conjugation of zearalenone and its reduced metabolites with glucuronic acid and sulfate occurs, mediated by catalysts such as uridine diphosphate-glucoronosyltransferases (UGTs) and sulfotransferases (SULTs). Based on in vitro studies, zearalenone and its metabolites are rapidly glucuronidated in the liver and intestine, but can also be metabolized in extrahepatic organs in both humans and several animal species (EFSA, 2011).
Excretion
Most mammals use bile and enterohepatic circulation for zearalenone excretion. The glucuronide derivatives of zearalenone are collected in the bile and again reabsorbed and metabolized in the intestinal mucosa. This reabsorption process contributes to the retention time of ZEA in the body, prolonging its toxic effect and hindering its elimination (Han et.al, 2022).
Ochratoxin
Chemical properties
Ochratoxins are composed of the union of a phenylalanine group with dihydroisocoumaric acid through a peptic bond, in which the carboxyl group (-COOH) and the amino group (-NH2) intervene. There are mainly two types of ochratoxins, A and B, which are distinguished by the presence of a chloride group in form A. Ochratoxins can be hydrolyzed, obtaining the metabolites OTalpha and OTbeta of lower toxicity.
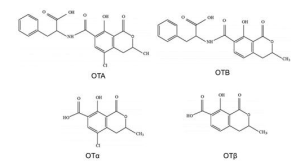
Figure 4. Chemical structure of the main ochratoxins and their metabolites.
Absorption
Ochratoxin is rapidly absorbed after oral ingestion, and it reaches high levels in blood for several hours. Passive diffusion through the stomach, and particularly the proximal region of the jejunum, results in the systemic entry of ochratoxin. Simultaneously this process is facilitated by the high affinity of plasma proteins with this toxin. The systemic bioavailability of the toxin changes depending on the species (Schrenk et.al, 2020).
Distribution
Regarding distribution, OTA is particularly relevant due to its strong non-covalent binding with serum proteins, especially albumin, which explains how difficult its subsequent elimination is and its long half-life in the body. OTA has its distribution in all organs of the body, however, the concentration that the toxin presents in each organ will depend on the animal species, the dose administered and how it has been studied (kinetic study design). Most studies or reports indicate that high concentrations of OTA are frequently expressed in the kidney, followed by the liver and muscle. Furthermore, redistribution and deposition of this toxin can occur in lipid-rich organs. Regarding the transfer of ochratoxin via the placenta, transport is minimal as indicated by certain in vitro studies. Furthermore, evidence suggests that OTA transfer is related to placental unwrapping or development. The transfer of the toxin can also occur through the uterine route or through breast milk in both animals and humans. Studies in dairy cows found traces of ochratoxin in milk, alluding that the minimum concentration of the toxin in this animal product can be attributed to the efficient presystemic degradation generated by the ruminal microflora (Schrenk et.al, 2020).
Metabolism
The principal metabolite of OTA is OTalpha, which is formed when the amide bridge between phenylalanine and dihydroisocoumaric acid is hydrolyzed. This molecule is generated by the intestinal microbiota in non-ruminant animals including humans, and in turn, in the ruminal microbiota of cows, sheep and other ruminants. OTA degradation is carried out by numerous hydrolases, such as carboxypeptidase A, which is the most active. The formation of OTalpha is considered an important step for the detoxification process and once this metabolite is generated, it does not accumulate in the kidney and is quickly excreted in the urine, as a glucuronide (Schrenk et.al, 2020).
Excretion
The complex binding of OTA to albumin results in reduced elimination of the toxin by glomerular filtration. Tubular secretion is the responsible for eliminating OTA by using organic anion transporters. Unfortunately, the toxin can be reabsorbed at tubular level and this phenomenon is partially responsible for its accumulation in the body. In addition, various in vivo studies have stated that the toxin has the ability to be reabsorbed in any part of the nephron, using active transport or passive diffusion according to the pH. Biliary excretion of OTA and its metabolites is the most important route through the fecal route. Similar to the urinary route, OTA can be absorbed again after being hydrolyzed by the bacterial microflora and continue in the enterohepatic circulation, whereupon it is slowly eliminated from the body. Finally, there is evidence that OTA can contaminate and be excreted in milk, where there is a direct relationship between the intake of OTA and its concentration in milk (Koszegi & Poor, 2016).
Toxin T-2
Chemical properties
Toxin T-2 is a mycotoxin that belongs to the trichothecene type A family. T-2 toxin is characterized by having two ester groups at carbons 4 and 8 in its molecular structure. Its main metabolite is HT-2 toxin, which is generated by the hydrolysis of the ester at carbon position 4 to a hydroxide group.
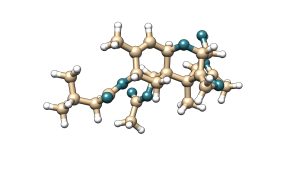
T-2
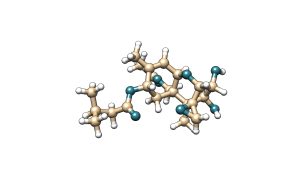
HT-2
Figure 5. Chemical structure of Toxin T-2 and its main metabolite HT-2.
Absorption
Toxin T-2 absorption is rapid after ingestion in most animal species, and it distributes throughout the body with little or no accumulation in a specific organ. It is a lipophilic toxin that, in addition to being absorbed through the gastrointestinal tract, can penetrate the respiratory mucosa (Janik et al., 2021).
Distribution
Toxin T-2 quickly moves from plasma to tissues or organs, with a plasma half-life of less than 20 minutes. It is distributed in organs such as the liver or kidney without significant accumulation. At the same time, T-2 has the ability to cross the placenta and fetal tissues (CONTAM, 2011).
Metabolism
The metabolism of T-2 toxin takes place in the intestine, liver and other tissues (CONTAM, 2011). The main metabolic pathways of this toxin include hydrolysis, hydroxylation, conjugation and depoxidation. The predominant metabolite of T-2 toxin in all species is HT-2, obtained as a result of the hydrolysis of the toxin. This metabolite is considered an important biomarker because it can detect the exposure of animals and humans to T-2 toxin (Janik et al., 2021). Most metabolites, especially T-2 toxin, are extensively conjugated with glucuronides, which is one of the most relevant mechanisms of detoxification (CONTAM, 2011).
Excretion
The urinary and biliary tracts are the routes used for the excretion of T-2 toxin and its metabolites. Excretion is rapid, with a higher proportion in feces. It is known that enterohepatic recycling of T-2 toxin and its glucuronide-conjugated metabolites occurs (CONTAM, 2011).
Fumonisins
Chemical properties
Fumonisins present a molecular structure similar to a lipid chain. There are four types of fumonisins, with type B1 being the most toxic, and differing in the presence of alcohol groups at positions 5 and 10 of the chain. Additionally, they possess branchings through esters at carbons 14 and 15 positions.
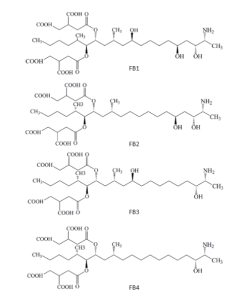
Figure 6. Chemical structure of the main fumonisins.
Absorption
Fumonisins are mycotoxins characterized by low bioavailability due to the charge of their structure and the limited expression of a specific receptor for their transport at the enteric level. In several species, reports indicate that fumonisins undergo a series of consecutive hydrolytic reactions mainly in the intestine, forming partially fumonisin A and fumonisin B and finally HFB1 or aminopentol. In exposed animals, metabolites can be found in the liver, kidney, and to a lesser extent, the muscle (Schrenk et al., 2022).
Distribution
In numerous animal species, the distribution of fumonisin B1 after absorption in the gastrointestinal tract occurs preferably in the liver, kidney, and muscle. Through various reports, it is known that the liver and kidney show some sensitivity to fumonisin B1, which may be due to or correlate with the concentration of the toxin in the tissue after absorption (Knutsen et al., 2018).
Metabolism
The primary sites for fumonisin metabolism are the liver and the digestive tract, where the fundamental metabolic pathways are hydrolysis, acylation, and transamination. The main metabolite of fumonisin B1 is HFB1 or also called AP1. This is generated by the hydrolysis of the side chains of tricarballylic acid at carbons 14 and 15, which are then replaced by hydroxyl groups (Wang et al., 2015).
Excretion
Most studies in experimental and farm animals report that after oral ingestion of FB1 and FB2, these are excreted unaltered in the feces, and to a lesser extent, in the urine. Toxin excretion occurs in the first 12 and 48 hours via the urinary and fecal routes, respectively (Knutsen et al., 2018).
In conclusion, the toxicokinetics of mycotoxins is complex topic as differences between toxins and animal species are investigated and compared. Generally, mycotoxins tend to share mechanisms of absorption, distribution, metabolism, and excretion; however, the way they use different routes varies between species. Despite the difficulty in understanding them, there is no doubt that knowing mycotoxins behavior in the organism is crucial for developing strategies to mitigate their effects on the target organs of the organism.